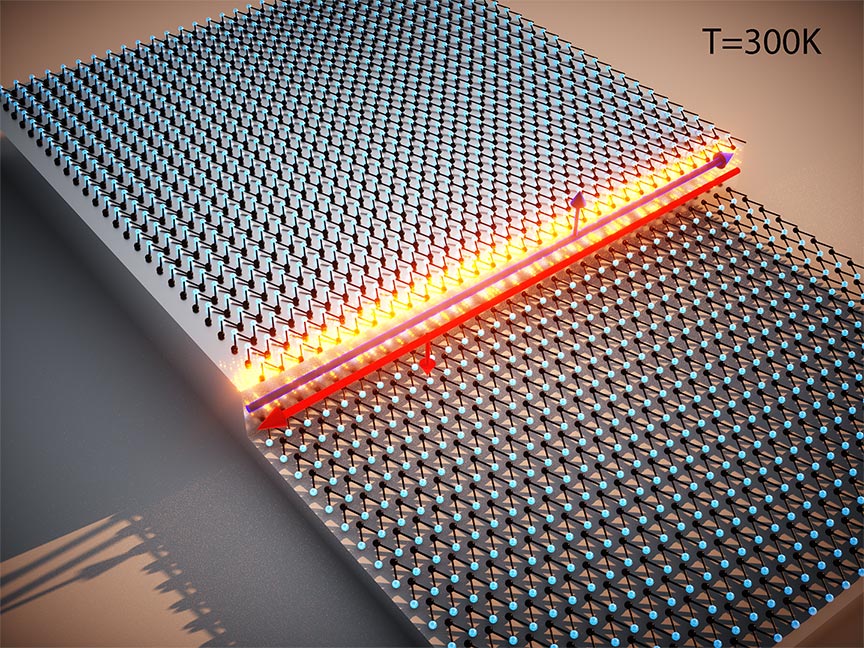
Investigadores de la Universidad de Princeton han descubierto que un material conocido como aislante topológico, hecho de los elementos bismuto y bromo, exhibe comportamientos cuánticos especializados que normalmente solo se observan en condiciones experimentales extremas de altas presiones y temperaturas cercanas al cero absoluto. Crédito: Shafayat Hussain y Muhammad Zahid Hassan de la Universidad de Princeton
Por primera vez, los físicos han observado nuevos efectos cuánticos en un aislador topológico a temperatura ambiente.
Investigadores en[{» attribute=»»>Princeton University discovered that a material known as a topological insulator, made from the elements bismuth and bromine, exhibits specialized quantum behaviors normally seen only under extreme experimental conditions of high pressures and temperatures near absolute zero. The finding opens up a new range of possibilities for the development of efficient quantum technologies, such as spin-based, high-energy-efficiency electronics.
Physicists have observed novel quantum effects in a topological insulator at room temperature for the first time. This breakthrough came when scientists from Princeton University explored a topological material based on the element bismuth. The study was published as the cover article of the October issue of the journal Nature Materials.
While scientists have used topological insulators to demonstrate quantum effects for more than a decade, this experiment is the first time these effects have been observed at room temperature. Inducing and observing quantum states in topological insulators typically requires temperatures around absolute zero, which is equal to minus 459 degrees Fahrenheit (or -273 degrees Celsius).
This finding opens up a new range of possibilities for the development of efficient quantum technologies, such as spin-based electronics, which have the potential to replace many current electronic systems with substantially higher energy efficiency.
In recent years, the study of topological states of matter has attracted considerable attention among physicists and engineers. In fact, it is presently the focus of much international interest and research. This area of study combines quantum physics with topology — a branch of theoretical mathematics that explores geometric properties that can be deformed but not intrinsically changed.

M. Zahid Hasan. Credit: Princeton University
“The novel topological properties of matter have emerged as one of the most sought-after treasures in modern physics, both from a fundamental physics point of view and for finding potential applications in next-generation quantum engineering and nanotechnologies,” said M. Zahid Hasan, the Eugene Higgins Professor of Physics at Princeton University, who led the research. “This work was enabled by multiple innovative experimental advances in our lab at Princeton,” added Hasan.
A topological insulator is the main device component used to investigate the mysteries of quantum topology. This is a unique device that acts as an insulator in its interior, which means that the electrons inside are not free to move around and therefore do not conduct electricity. However, the electrons on the device’s edges are free to move around, meaning they are conductive. Moreover, because of the special properties of topology, the electrons flowing along the edges are not hampered by any defects or deformations. This device has the potential not only of improving technology but also of generating a greater understanding of matter itself by probing quantum electronic properties.
Until now, however, there has been a major stumbling block in the quest to use the materials and devices for applications in functional devices. “There is a lot of interest in topological materials and people often talk about their great potential for practical applications,” said Hasan, “but until some macroscopic quantum topological effect can be manifested at room temperature, these applications will likely remain unrealized.”
This is because ambient or high temperatures create what physicists call “thermal noise,” which is defined as a rise in temperature such that the atoms begin to vibrate violently. This action can disrupt delicate quantum systems, thereby collapsing the quantum state. In topological insulators, in particular, these higher temperatures create a situation in which the electrons on the surface of the insulator invade the interior, or “bulk,” of the insulator, and cause the electrons there to also begin conducting, which dilutes or breaks the special quantum effect.
The way around this is to subject such experiments to exceptionally cold temperatures, typically at or near absolute zero. At these incredibly low temperatures, atomic and subatomic particles cease vibrating and are consequently easier to manipulate. But creating and maintaining an ultra-cold environment is impractical for many applications; it is costly, bulky, and consumes a considerable amount of energy.
However, Hasan and his team have developed an innovative way to bypass this problem. Building on their experience with topological materials and working with many collaborators, they fabricated a new kind of topological insulator made from bismuth bromide (chemical formula α-Bi4Br4), which is an inorganic crystalline compound sometimes used for water treatment and chemical analyses.
“This is just terrific that we found them without giant pressure or an ultra-high magnetic field, thus making the materials more accessible for developing next-generation quantum technology,” said Nana Shumiya, who earned her Ph.D. at Princeton, is a postdoctoral research associate in electrical and computer engineering, and is one of the three co-first authors of the paper.
She added, “I believe our discovery will significantly advance the quantum frontier.”
The discovery’s roots lie in the workings of the quantum Hall effect — a form of topological effect that was the subject of the Nobel Prize in Physics in 1985. Since that time, topological phases have been intensely studied. Many new classes of quantum materials with topological electronic structures have been found, including topological insulators, topological superconductors, topological magnets, and Weyl semimetals.
While experimental discoveries were rapidly being made, theoretical discoveries were also progressing. Important theoretical concepts on two-dimensional (2D) topological insulators were put forward in 1988 by F. Duncan Haldane, the Sherman Fairchild University Professor of Physics at Princeton. He was awarded the Nobel Prize in Physics in 2016 for theoretical discoveries of topological phase transitions and a type of 2D topological insulators. Subsequent theoretical developments showed that topological insulators can take the form of two copies of Haldane’s model based on electron’s spin-orbit interaction.
Hasan and his team have been on a decade-long search for a topological quantum state that may also operate at room temperature, following their discovery of the first examples of three-dimensional topological insulators in 2007. Recently, they found a materials solution to Haldane’s conjecture in a kagome lattice magnet that is capable of operating at room temperature, which also exhibits the desired quantization.
“The kagome lattice topological insulators can be designed to possess relativistic band crossings and strong electron-electron interactions. Both are essential for novel magnetism,” said Hasan. “Therefore, we realized that kagome magnets are a promising system in which to search for topological magnet phases, as they are like the topological insulators that we discovered and studied more than ten years ago.”
“A suitable atomic chemistry and structure design coupled to first-principles theory is the crucial step to make topological insulator’s speculative prediction realistic in a high-temperature setting,” said Hasan. “There are hundreds of topological materials, and we need both intuition, experience, materials-specific calculations, and intense experimental efforts to eventually find the right material for in-depth exploration. And that took us on a decade-long journey of investigating many bismuth-based materials.
Insulators, like semiconductors, have what are called insulating, or band, gaps. These are in essence “barriers” between orbiting electrons, a sort of “no-man’s-land” where electrons cannot go. These band gaps are extremely important because, among other things, they provide the lynchpin in overcoming the limitation of achieving a quantum state imposed by thermal noise. They do this if the width of the band gap exceeds the width of the thermal noise. But too large a band gap can potentially disrupt the spin-orbit coupling of the electrons — this is the interaction between the electron’s spin and its orbital motion around the nucleus. When this disruption occurs, the topological quantum state collapses. Therefore, the trick in inducing and maintaining a quantum effect is to find a balance between a large band gap and the spin-orbit coupling effects.
Following a proposal by collaborators and co-authors Fan Zhang and Yugui Yao to explore a type of Weyl metals, Hasan and his team studied the bismuth bromide family of materials. But the researchers were not able to observe the Weyl phenomena in these materials. They instead discovered that the bismuth bromide insulator has properties that make it more ideal compared to a bismuth-antimony-based topological insulator (Bi-Sb alloys) that they had studied before. It has a large insulating gap of over 200 meV (“milli electron volts”). This is large enough to overcome thermal noise, but small enough so that it does not disrupt the spin-orbit coupling effect and band inversion topology.
“In this case, in our experiments, we found a balance between spin-orbit coupling effects and large band gap width,” said Hasan. “We found there is a ‘sweet spot’ where you can have relatively large spin-orbit coupling to create a topological twist as well as raise the band gap without destroying it. It’s kind of like a balance point for the bismuth-based materials that we have been studying for a long time.”
When the researchers viewed what was going on in the experiment through a sub-atomic resolution scanning tunneling microscope, they knew they had achieved their goal. This microscope is a unique device that uses a property known as “quantum tunneling,” where electrons are funneled between the sharp metallic, single-atom tip of the microscope and the sample. The microscope uses this tunneling current rather than light to view the world of electrons on the atomic scale. The team observed a clear quantum spin Hall edge state, which is one of the important properties that uniquely exist in topological systems. This required additional novel instrumentation to uniquely isolate the topological effect.
“For the first time, we demonstrated that there’s a class of bismuth-based topological materials that the topology survives up to room temperature,” said Hasan. “We are very confidant of our result.”
This finding is the culmination of many years of hard-won experimental work and required additional novel instrumentation ideas to be introduced in the experiments. Hasan has been a leading researcher in the field of experimental quantum topological materials with novel experimentation methodologies for over 15 years; and, indeed, was one of the field’s early pioneer researchers. Between 2005 and 2007, for example, he and his team of researchers discovered topological order in a three-dimensional bismuth-antimony bulk solid, a semiconducting alloy and related topological Dirac materials using novel experimental methods. This led to the discovery of topological magnetic materials. Between 2014 and 2015, they discovered a new class of topological materials called magnetic Weyl semimetals. The researchers believe this breakthrough will open the door to a whole host of future research possibilities and applications in quantum technologies.
“We believe this finding may be the starting point of future development in nanotechnology,” said Shafayat Hossain, a postdoctoral research associate in Hasan’s lab and another co-first author of the study. “There have been so many proposed possibilities in topological technology that await, and finding appropriate materials coupled with novel instrumentation is one of the keys for this.”
One area of research where Hasan and his team believe this breakthrough will have particular impact is on next-generation quantum technologies. The researchers believe this new breakthrough will hasten the development of more efficient, and “greener” quantum materials.
Currently, the theoretical and experimental focus of the group is concentrated in two directions, said Hasan. First, the researchers want to determine what other topological materials might operate at room temperature, and, importantly, provide other scientists the tools and novel instrumentation methods to identify materials that will operate at room and high temperatures. Second, the researchers want to continue to probe deeper into the quantum world now that this finding has made it possible to conduct experiments at higher temperatures.
These studies will require the development of another set of new instrumentations and techniques to fully harness the enormous potential of these materials. “I see a tremendous opportunity for further in-depth exploration of exotic and complex quantum phenomena with our new instrumentation, tracking more finer details in macroscopic quantum states,” Hasan said. “Who knows what we will discover?”
“Our research is a real step forward in demonstrating the potential of topological materials for energy-saving applications,” added Hasan. “What we’ve done here with this experiment is plant a seed to encourage other scientists and engineers to dream big.”
Reference: “Evidence of a room-temperature quantum spin Hall edge state in a higher-order topological insulator” by Nana Shumiya, Md Shafayat Hossain, Jia-Xin Yin, Zhiwei Wang, Maksim Litskevich, Chiho Yoon, Yongkai Li, Ying Yang, Yu-Xiao Jiang, Guangming Cheng, Yen-Chuan Lin, Qi Zhang, Zi-Jia Cheng, Tyler A. Cochran, Daniel Multer, Xian P. Yang, Brian Casas, Tay-Rong Chang, Titus Neupert, Zhujun Yuan, Shuang Jia, Hsin Lin, Nan Yao, Luis Balicas, Fan Zhang, Yugui Yao and M. Zahid Hasan, 14 July 2022, Nature Materials.
DOI: 10.1038/s41563-022-01304-3
The team included numerous researchers from Princeton’s Department of Physics, including present and past graduate students Nana Shumiya, Maksim Litskevich, Yu-Xiao Jiang, Zi-Jia Cheng, Tyler Cochran and Daniel Multer, and present and past postdoctoral research associates, Shafayat Hossain, Jia-Xin Yin and Qi Zhang. Other co-authors were Zhiwei Wang, Chiho Yoon, Yongkai Li, Ying Yang, Guangming Cheng , Yen-Chuan Lin, Brian Casas, Tay-Rong Chang, Titus Neupert , Zhujun Yuan, Shuang Jia , Hsin Lin and Nan Yao .
The work at Princeton was supported by the U.S. Department of Energy’s Basic Energy Sciences Division (and the Gordon and Betty Moore Foundation’s Emergent Phenomena in Quantum Systems Initiative.